Interfacial and Material Energetics
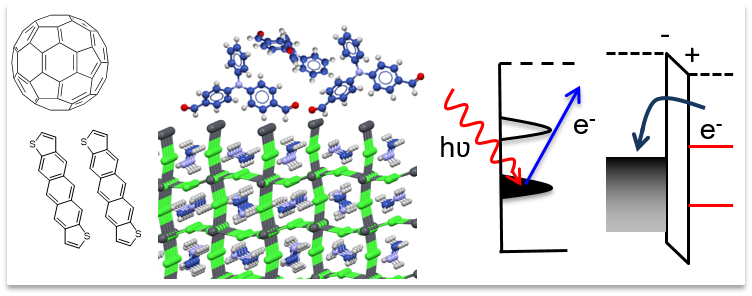
Interfacial and material energetics are a common thread throughout our research. We aim to understand surface chemistries, how these chemistries influences interfacial energy landscapes, and how these interfacial energy landscapes influence device and material properties. We aim to apply our findings to develop new and improved materials and devices. The sections below describe our two primary application oriented research directions: solar cells and thermoelectrics.
To understand and utilize interfacial and material energy landscapes, we must first be able to measure them. Here, we primarily utilize X-ray, ultraviolet, and inverse photoemission spectroscopies (XPS, UPS, and IPES, respectively). This is particularly challenging for organic materials, as many commonly used organic semiconductors are damaged by the relatively high photon flux from commonly used laboratory-based He I discharge sources in UPS and the high-energy electrons in IPES. To reduce this problem of sample damage we have adopted a low-energy (10.2 eV) photon source with a photon flux that can be adjusted over a 4 order of magnitude range and a low-energy IPES setup that utilizes a photomultiplier tube with a bandpass filter as the low-energy photon detector. These customized measurement setups are both contained in a single ultra-high vacuum (UHV) chamber that is directly connected to our thermal evaporator and glovebox. This direct connection allows us to deposit materials in angstrom level thicknesses and take UPS and IPES measurements of the energetic landscape as the interface is being formed. For solution processed films, this direct connection between the glovebox and UHV chamber allows us to probe energy levels in the absence of air exposure.
Composite Thermoelectrics
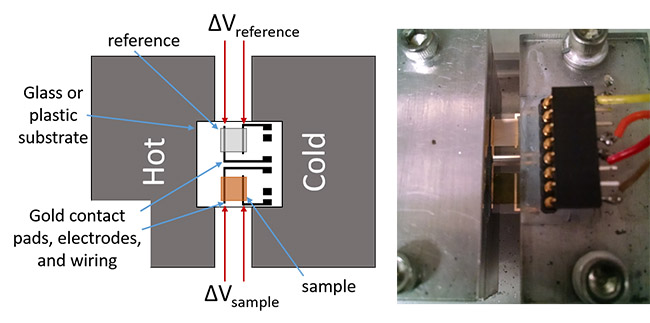
Thermoelectrics have two primary applications. The Seebeck effect can be used to convert heat into electricity, while the Peltier effect can be utilized for refrigeration and other cooling applications. Traditional thermoelectrics are made from brittle materials that typically contain relatively expensive and rare elements, which is fine for use where cost is not an issue or where a significant amount of energy can be recovered (i.e. from high temperature differentials). However, for more widespread use and use in applications where a flexible form factor is desired, alternatives to traditional inorganic materials are necessary. Currently, over half of the raw energy used in the world is wasted in the form of heat. If a small fraction of this currently wasted thermal energy can be recovered it will have a huge impact on overall energy consumption. It is these low-cost alternative thermoelectric materials that may enable widespread applications in recovering waste heat energy, or in enabling the development of low-cost refrigeration.
In general, thermoelectrics rely on the spontaneous flow of entropy from the hot to the cold side (Seebeck effect) of a material, or the electrically powered flow of entropy from the cold side to the hot side (Peltier effect). In an efficient thermoelectric material, a significant amount of entropy is transported by charge-carriers, as opposed to phonons. The performance of these materials depend heavily on the energy dependence of charge transport. Manipulating this energy dependence of charge transport provides a route to developing improved thermoelectric materials. Recent work has shown relatively high thermoelectric performance for materials based on conducting polymers and conducting polymer-inorganic composites. These materials have the potential for high thermoelectric performance utilizing low-cost processing methods and materials while producing flexible devices, but currently their efficiencies are 5 to 10 fold lower than desired for commercial applications. Here, our focus is largely on how interfacial energetics and charge-transfer rates influence the performance of these organic-inorganic composites. We are working on the synthesis and surface modification of nanoparticles and nanowires, experimentally measuring the effects of surface chemistry on energetics and transport, and incorporating these nanoparticles and wires into composites to determine how film morphology and energetics effect the electrical conductivity and the Seebeck coefficient. It is expected that creating appropriate energy landscapes and high charge-transfer rates in these nanocomposites will provide a route to high-performing thermoelectric materials. In addition, we are pursuing a similar strategy with polymer blend thermoelectrics. Here, two pi-conjugated polymers with different energetics and electrical transport behavior are being investigated.
Solar Cells
Solar cells are playing an increasing role in electricity generation, and to be more economically feasible at a large scale we need ways of making them quicker and cheaper. Two emerging material systems that are likely to enable inexpensive solution-processed solar cells with high performance are pi-conjugated organics and organometal halide based perovskites, with our current research efforts centered more on organometal halide perovskites. Perovskite solar cells are the most rapidly emerging solar cell technology, with efficiencies climbing from less than 5% to over 20% in the last 9 years. In addition to their use in solar cells, these perovskites can also be used in low-cost solid-state light emitting diodes (LEDs). Like any new electronic material system, these perovskite containing solar cells and LEDs still face their share of challenges. In part, these include obtaining reproducibly high efficiencies, replacing lead with a less toxic metal, obtaining long operating lifetimes, extending the absorbance further into the near-IR for tandem solar cells, and developing solution-processable and flexible electrodes and interlayers to enable completely solution-processed devices on flexible substrates. A major aspect of our research on perovksites is in understanding the surface chemistry and in using surface modifiers to alter interfacial energetics, trap states, and stability. To accomplish this we include a host of analytical measurements, including our customized photoelectron spectrocopy measurements. Building on our focus on interfaces and surface chemistry, another focal area is in the development of improved electron and hole transport layers. Here, we aim to develop a better understanding of how the perovskite/transport layer interfacial chemistry and energetics influence charge recombination dynamics and overall solar cell performance. We are currently addressing these aspects in both high-efficiency lead containing perovksites and lower efficiency, but less toxic, tin containing perovksites.